Composite Materials
Main Content
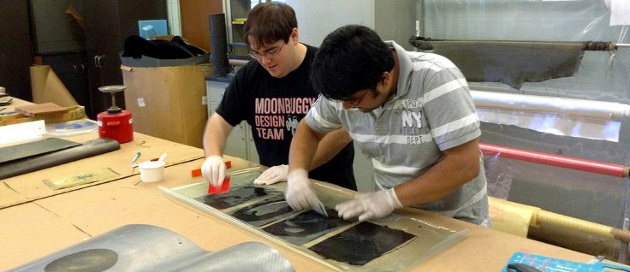
Primarily developed for military aerospace/aircraft applications, advanced composites such as Carbon-Carbon (C/C) composites, Carbon Fiber Reinforced Plastics (CFRP), and honeycomb sandwiched panels are now widely used in many commercial applications such as aerospace, automotive, bridges, railroads, gas and liquid transmission pipelines, and medical and marine industries. The main reason for this is that composites provide a higher strength-to-weight ratio and good stiffness properties compared to traditional metals and their alloys. With advanced composites being significantly used today in variety of applications, being able to test them for flaws has been more pertinent than ever before. Also, detection of defects in composites is very important both for quality control during the production process and during in-service inspection.
IMEL research on composites primarily focuses on the NDE of Carbon-Carbon (C/C) composites, graphite/epoxy composites, composite repairs, and developing an automated procedure for the detection and characterization of defects.
Carbon-Carbon (C/C) Composites
C/C composites are made from carbon fibers reinforced in a carbonaceous matrix by a lengthy and expensive manufacturing process that involves exposure to extreme heat (pyrolysis) and vacuum pressure (Fitzer, 1987; Fitzer and Manocha, 1985; Savage, 1993). The material properties of C/C composites greatly depend on the fiber selection and orientation, as well as the fabrication process, which is highly proprietary in the C/C industry. The precursor materials for carbon fibers considered for producing C/C brake disks include: polyacrylonitrile (PAN), pitch or tar. Today, more than 90% of the commercially marketed carbon fibers are made from PAN, which offers a higher strength-modulus and failure strain with high char yield compared to fibers made from pitch or rayon (Fitzer, 1987; Savage, 1993).
Today, approximately 63% by volume of the C/C produced in the world are used in aircraft braking systems (Savage, 1993). The main reason for this is that C/C disks offer outstanding thermal and physical properties, which include: light weight compared to metals, high thermal shock resistance, low coefficient of thermal expansion, retention of strength at higher temperatures, high impact resistance, and high stiffness (Fitzer, 1987; Fitzer and Manocha, 1985). Carbon-carbon (C/C) composite brake disks are widely used today as the most effective friction material for many high speed automotive and aircraft applications. The brakes are usually designed to dissipate the kinetic energy into heat during the braking condition. Approximately 450 MJ of energy or more than 1000 kN m of torque must be absorbed and dissipated by the rotors and stators of a fully loaded (maximum mass of 158 000 kg) jet airliner’s brake assemblies during landing.
In one manufacturing approach, commercial 2D random fiber C/C brake disks are made with towpregs (a mixture of random chopped carbon fiber/phenolic bonded resins) compacted by hot pressing in an annular mold to produce a disk shaped preform.
The carbon fiber reinforced phenolic bonded resin composites are then carbonized (organic components are removed) to convert them into C/C composites in an inert atmosphere, usually ultra-pure nitrogen, at controlled temperature ranging from 1273 to 1773 K (1000 to 1500 °C). This process is called carbonization or graphitization. During this stage, the polymer matrix decomposes. As a result, porosity and cracks form due to the release of vapor (primarily water) byproducts and the thermal expansion mismatch between the fiber and matrix. In addition, the preform loses more than half of its initial weight, but its carbon content increases from 65% to 99% (Fitzer, 1987; Savage, 1993). The resulting porous structure, however, has poor physical properties and therefore requires further processing steps, typically chemical vapor infiltration (CVI).
The CVI process is carried out at high temperatures of approximately 1273 K (1000 °C), causing the carbon preform to become densified. During this process, the pyrolysis of hydrocarbon gas takes place, and pyrolytic carbon or chemical vapor deposition carbon is deposited on the walls of open porosity and on the carbon fibers. This process is repeatedly carried out until a desired density (approximately1.7 mg/mm3) of the C/C is reached. After the material properties of the C/C brake disks are met, the disks are machined to their final dimensions and heat-treated if high thermal properties are desired (Ozcan et al., 2009). Heat treatment can drastically increase the thermal diffusivity and thermal conductivity of the C/C composites. Heat treatment can range from 1873 to 2773 K (1600 to 2500 °C). The higher the temperature, the greater the thermal conductivity and diffusivity values that can be obtained; but, higher temperature may impact the friction properties. On the other hand, heat treatment can lead to the formation of internal discontinuities, predominantly cracks (Ozcan et al., 2009). In some cases, further CVI is performed to fill in the newly opened porosity from the heat treatment and the form may be heat treated again.
Two-dimensional C/C has low interlaminar shear strength, making it prone to delaminations if processing steps are not carried out properly (Fitzer and Manocha, 1985). It becomes difficult to identify delaminations, cracks, and porosity (resin pockets) at the early stages of the manufacturing process. To ensure the safety and reliability of C/C brake disks right after the manufacturing process, IMEL has explored and successfully applied thermal, ultrasonic, and radiographic nondestructive testing (NDT) techniques.
Graphite/Epoxy Composites
Graphite/epoxy composites or carbon fiber reinforced plastics (CFRP) have been around for many years and their material properties have improved significantly. These composites are made up of one or more layers depending on the application requirements. There are two main types of carbon fiber patterns that use rovings: unidirectional and woven. The unidirectional CFRP lines a layer of fibers parallel to each other, then the next layer will be the same but will have a different orientation compared to the first layer and the layers will continue to change orientation as more layers are added. In general, a simple woven pattern would have fibers going in one direction and then another set of fibers perpendicular, woven in between the first set of fibers in the same layer. Depending on the needed parameters, many layers are put on top of each other making a panel or some other desired shape. An epoxy or thermoplastic based resin will be used to bond the fibers together, and is referred to as the matrix. A tighter weave will add stiffness and strength to the panel but will be more brittle whereas a loose weave will be inversely proportional. Also, the type of matrix used will dictate how the panel acts under different loading conditions. The manufacturing process of CFRP is very complicated and its material properties greatly depend on the fiber selection and orientation, as well as the fabrication process, which is therefore highly proprietary in the CFRP industries.
However, CFRPs are prone to damage mechanisms which can occur during the processing stages or during in-service operations. Some of the flaws that are often encountered include delaminations, cracks, disbonds, impact damage, porosity, inclusions, matrix cracking, fiber breakage, fiber wrinkling or waviness, and fiber and ply misalignment. Several NDE methods are often employed to inspect and evaluate several composite structures during the production process or during in-service operations. IMEL primarily focuses on the use of infrared thermography, conventional ultrasonic, and acoustography NDE techniques for the inspection of composite laminates.
Composite Repairs
Composite structures are primarily damaged by lightning strikes, hail damage, low/high velocity impacts caused by runway debris and birds, tool drops, and service vehicle collisions. Other sources of damage include erosion, abrasion, manufacturing defects, excessive heat exposure, and fluid infiltration (Tomblin, et al., 2004). Sometimes damage to composite components may not be visible to the naked eye. Therefore, various non-destructive evaluation (NDE) methods are often applied today to identify, characterize these defects and evaluate the extent of damages in structural components. In most cases, replacing the entire part is not economically feasible; thus, repairing it is the only viable solution. Furthermore, since the time constraint is an issue, repairs must be performed as quickly as possible so that the aircraft can be returned to service as soon as possible.
The standards for repair on sheet metal aircrafts are well known and documented with published substitutions and variances. Typically, repair technicians have a supply of rivets, fasteners, and various sheets of metal on hand to mend most damaged metal in aircrafts. But, the adhesively bonded repair of damaged composite sandwich structures is a complex problem, because several factors must be considered to ensure the repair’s effectiveness and structural integrity. Some of these factors include stiffness, strength, stability, operating temperature, durability, and aerodynamic smoothness.
With the increase of composite aircraft there is a requirement for standard and effective repair procedures. Several aircraft manufacturers have established unique repair procedures specifically for each type of design based on their rigorous tests and analyses. Aircraft Maintenance Technicians (AMT) and inspectors are adapting their technology and training requirements to cope with these composite aircrafts. The repair techniques used in the old fiberglass shops on non-structural components are not suited for the advanced composites used today. Composite repair training centers are being established to provide suitable courses.